Form and Function: The Physiological Implications of the Anatomy of the Respiratory System
The Gas Exchanger
If the controller and ventilatory pump have accomplished their respective tasks, air arrives at the gas exchanger, which is composed of the alveoli and the pulmonary capillaries. The bulk flow seen in the airways gives way to diffusion as oxygen leaves the alveolus and enters the blood and carbon dioxide moves in the opposite direction. As with the other components of the respiratory system, nature has constructed a very efficient structure to perform this vital function.
Requirements of the Gas Exchanger β
Having moved air from the pharynx to the respiratory bronchioles, the system must now provide a large surface area for the diffusion of oxygen into and carbon dioxide out of the blood. In addition, to ensure efficient transfer of gas between the blood and the alveolus, the distance for diffusion must be as short as possible. The gas-exchanging unit, however, must be sufficiently robust to resist collapse during exhalation as the lung volume de- creases. Finally, the system must ensure that perfusion, the term given to blood flow to an organ or tissue bed, goes to the areas of the lung where oxygen is being delivered.
Large Surface Area for Diffusion β
The amount of gas that can dilfuse across a surlace is proportional to the area of the surface. To maximize the surlace area for gas exchange, millions of gas exchanging units are present at the ends of the respiratory bronchioles. These gas-exchanging units, or alveoli, can be found primarily as part of the alveolar ducts. A respiratory bronchiole, which also contains a few alveoli emanating from its wall, and its associated alveolar ducts are the site of gas exchange and constitute an acinus or pulmonary lobule. Roughly 300 million alveoli are present in the lung, each of which is less than 0.5 mm in diameter. The total surface area of the gas-exchanging units in the lungs is between 50 and 100 m2.
Because the surface area of the alveoli is large, a comparably large pulmonary capillary surface area is needed to achieve effective transfer of oxygen and carbon dioxide between the two structures. The pulmonary capillaries are literally wrapped around the alveolus a fine mesh network that produces a virtual sheet of blood for diffusion (Fig. 2-9).
The pulmonary capillaries form a fine mesh network around each alveolus to maximize the contact between the two structures and, consequently, the surface area for diffusion of gas. Each alveolus contains a few alveoli emanating from its wall, and its associated alveolar ducts are the site of gas exchange and constitute an acinus or pulmonary lobule. Roughly 300 million alveoli are present in the lung, each of which is less than 0.5 mm in diameter. The total surface area of the gas-exchanging units in the lungs is between 50 and 100 m^2.
As many as 1000 pulmonary capillaries come into contact with each alveolus. Since the diameter of a capillary is only 10 πm, a size that permits just a single RBC to pass through the vessel, the gas has only a minimal distance to travel between the alveolus and the hemoglobin contained within the RBC. Hemoglobin is the primary protein within the RBC and, by virtue of its capacity to hold oxygen, is the major means by which oxygen is transported in the blood. Each molecule of hemoglobin contains four binding sites for oxygen, and we characterize the extent to which the blood is "filled" with oxygen by measuring the percentage of binding sites that are bound with the molecule. This percentage is called the oxygen saturation.
During the time that an RBC traverses a pulmonary capillary, it may actually come into contact with several alveoli. Assuming a normal cardiac output in a resting individual, hemoglobin in an RBC is typically fully saturated with oxygen by the time it has made it 25% to 33% of the way through the pulmonary capillary. This means that there is tremendous reserve capacity for diffusion. For example, when cardiac output is increased, as in exercise, and the speed with which an RBC traverses the alveolus increases, the blood will still be saturated with oxygen by the time it exits the capillary. Alternatively, if a disease process results in a thickening of the alveolar-capillary interface, there may not be adequate time for the oxygen to diffuse into the RBCs.
Under normal conditions, the walls of the alveolus and the capillary are so thin that the pressure within the alveolus can affect blood flow within the capillary. In some regions of the lung, pressure in the alveolus exceeds that within the capillary, leading to compression or collapse of the capillary (more in Chapter 5).
Minimizing Distance for Diffusion β
The amount of gas that can diffuse across a surface is inversely proportional to the thickness of the surface. The total distance for diffusion of gas from the alveolus to the RBC is approximately 0.5 πm. The surface across which the gas must diffuse consists of the fluid lining the alveolus, the alveolar wall, the interstitial space between the alveolus and the capillary (a space that is negligible in normal individuals but where fluid and inflammatory material may accumulate in disease states), the wall of the capillary, the plasma surrounding the RBC, and the wall of the RBC.
The wall of the pulmonary capillary is composed of a single layer of squamous epithelium, which minimizes the distance for diffusion. The wall of the alveolus is similarly thin, composed of an epithelium made up of a single layer of cells called type I pneumocytes and an interstitium formed by the fused basal lamina of the alveolar epithelium and the capillary endothelium (in some regions, collagen and elastin also contribute to the thickness of the interstitial space). In healthy people, the thickness of the RBCs forms a significant portion of the distance across which oxygen and carbon dioxide must diffuse.
Diffusion is so effective in the lungs, and the reserve time available for diffusion of oxygen into the RBCs is so great, that a low blood oxygen level attributable to diffusion limitation is difficult to demonstrate in the normal lung. In experimental conditions, a low blood oxygen level may occur in a person doing heavy exercise while breathing a mixture of gas with a low partial pressure of oxygen. Even in disease states, diffusion limitation is rarely a cause of hypoxemia, the term used for low blood oxygen levels at rest (see Chapter 5).
Stabilizing the Gas-Exchanging Unit β
As the volume of alveoli decreases during expiration, surface forces and the physics of small, gas-containing objects increase the probability that the alveoli will collapse (see Chapter 3). Anatomically, the alveoli are linked in ways that minimize the probability of collapse. Connective tissue, composed of collagen and elastin, is interspersed between the alveoli and the airways. As a consequence of this "connectedness," forces imposed at the level of the alveolar wall are transmitted to adjacent alveoli and the rest of the lung, thereby providing a form of stabilization to individual gas-exchanging units. Play Animated Figure 2-10 to view how the collapse of a single alveolus is opposed by its connections to surrounding alveoli.
π¬ Animated Figure 2-10 Stabilization of the gas-exchanging unit
Alveoli are linked to each other via shared walls and to airways via collagen and elastin. These connections allow forces to be shared among alveoli, and the resulting stabilization makes it difficult for a single alveolus to collapse. This form of structural support is also known as "interdependence." Play the animated figure to view how the collapse of a single alveolus is opposed by its connections to surrounding alveoli.
The wall of one alveolus is often shared by another alveolus. If one alveolus were to collapse, therefore, others would be affected as the adjacent alveoli are pulled down in the same direction. The phenomenon of atelectasis, or collapse of lung units, is not caused collapse of individual alveoli; larger lung units must be involved given this degree of interdependence.
Matching Ventilation and Blood Flow β
For gas exchange to be optimized, the body must match the distribution of ventilation, or movement of air into the lung, with perfusion, the blood flow in the pulmonary capillaries. The pulmonary arteries are located next to the bronchi and branch with them down to the level of the respiratory bronchiole. Here, the arteries divide into capillaries that surround the alveoli, as already described. The pulmonary arteries, however, are not passive conduits for blood flow. The arterial walls contain smooth muscle that can contract, thereby changing the resistance of the vessel and the amount of blood flowing through a particular region of lung. Hypoxia is one of the stimuli that cause pulmonary arterial vasoconstriction. Thus, if one section of lung is not receiving adequate ventilation and local hypoxia develops in that area, the pulmonary artery serving that region will constrict to redirect blood flow to another area that is receiving adequate ventilation (Fig. 2-11). This phenomenon, termed hypoxic pulmonary vasoconstriction, ensures that blood flows to regions of lung receiving the best ventilation.
Figure 2-11 Hypoxic pulmonary vasoconstriction
Arterial smooth muscle cells in pulmonary arterioles respond to hypoxia by contracting. A) Baseline state with normally ventilated alveoli. B) Moderate airflow obstruction, with reduced PO2 in the corresponding alveolus and vasoconstriction of the associated pre-capillary vessels. C) More severe airflow obstruction, with low PO2 and stronger pre-capillary vasoconstriction. Hypoxic vasoconstriction matches ventilation to perfusion by redirecting blood flow (perfusion) to areas with higher ventilation.
A
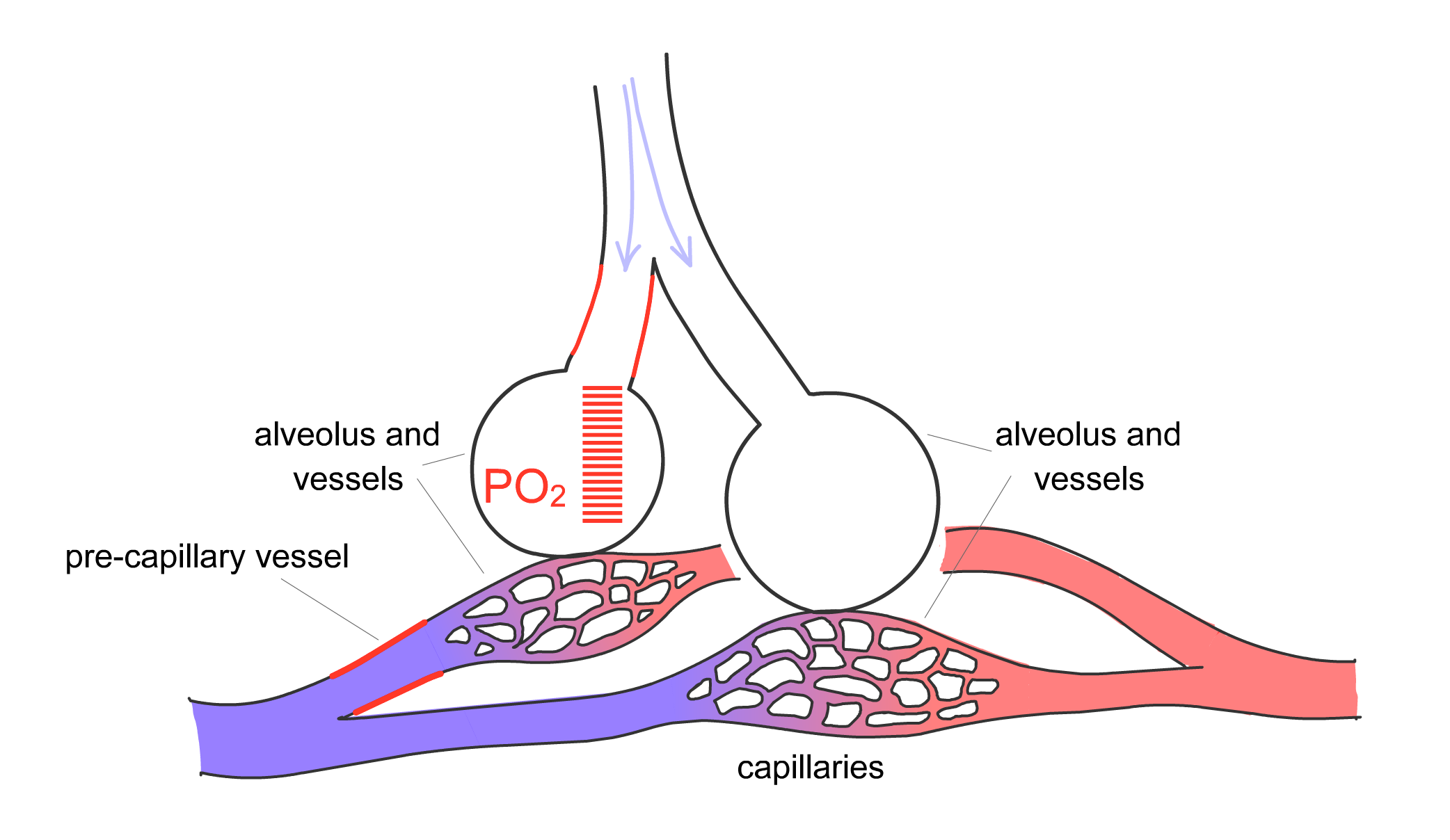
B
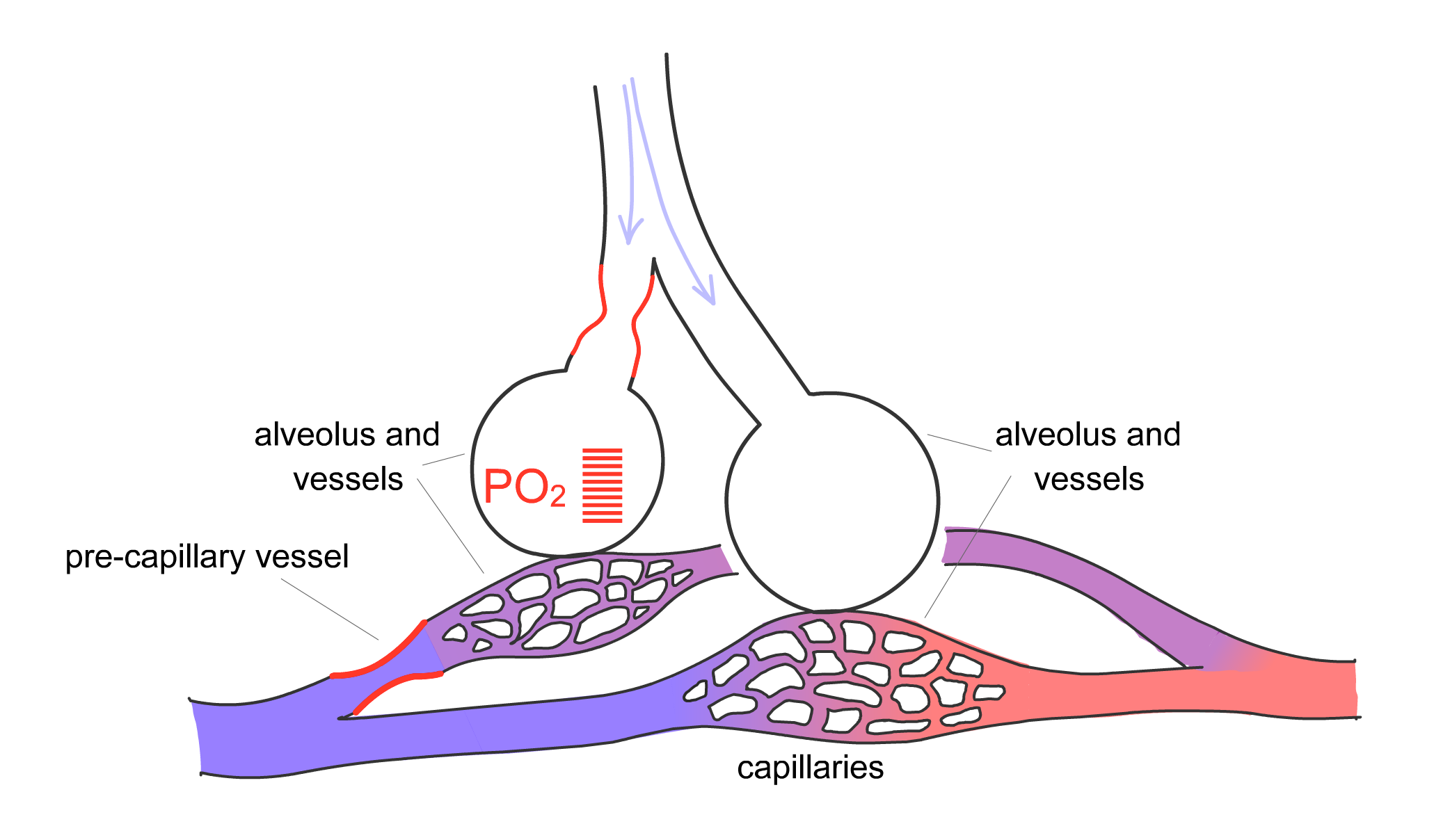
C
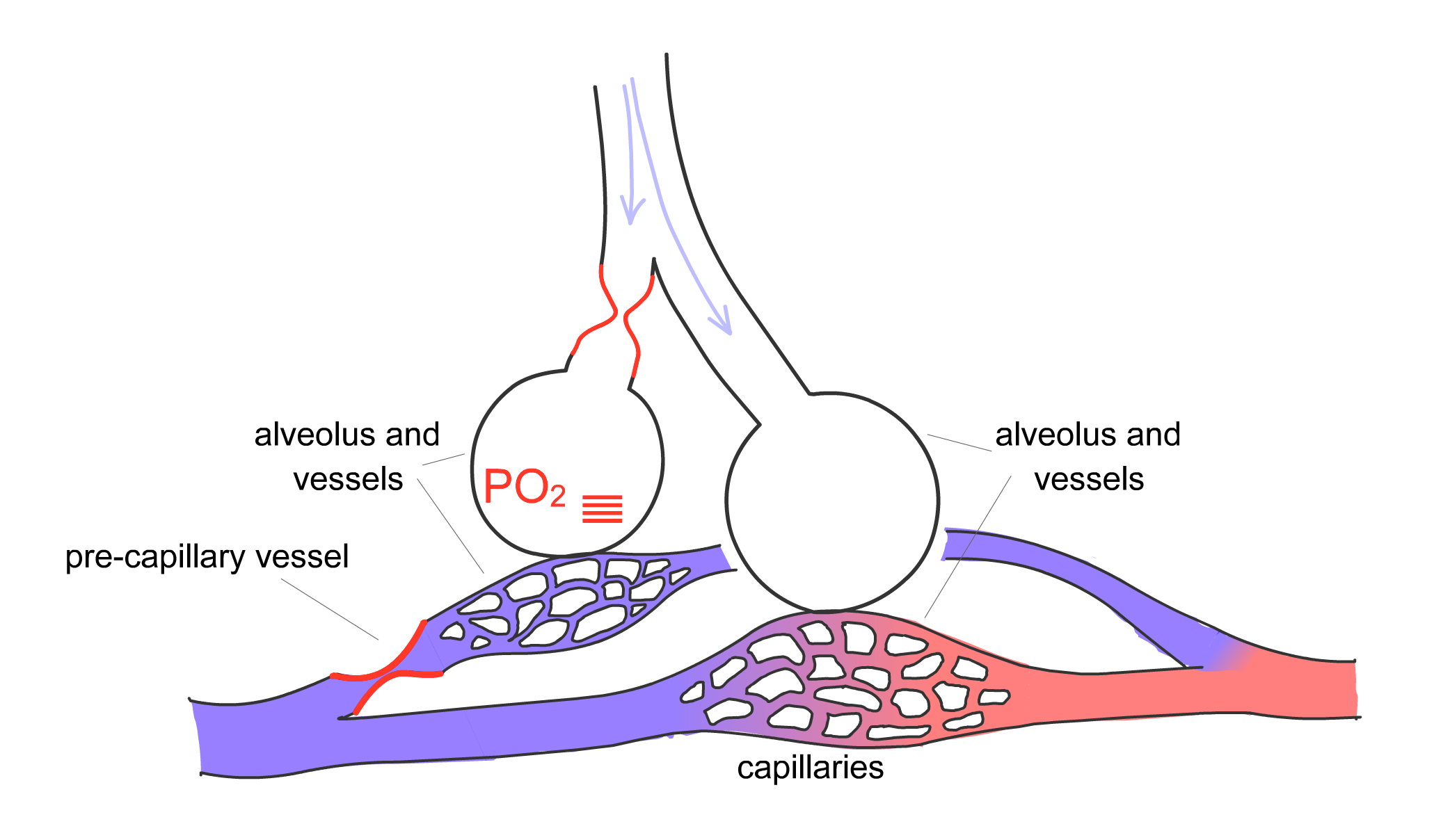
Play Animated Figure 2-11 to better understand the process of hypoxic vasoconstriction. Note that as alveolar hypoxia develops (i.e., its partial pressure of oxygen, or PO2, decreases), constriction of the pre-capillary vessel supplying the affected alveolus occurs, and blood flow is redirected to unaffected alveoli. By matching ventilation and perfusion, the body maximizes the opportunity for diffusion of oxygen and carbon dioxide. Mismatch of ventilation (represented by a V with a dot over it,
π¬ Animated Figure 2-11 Hypoxic pulmonary vasoconstriction
Arterial smooth muscle cells in pulmonary arterioles respond to hypoxia by contracting. As ventilation decreases to one alveolus, the partial pressure of oxygen diminishes within that unit. This process leads to an increase in vascular resistance in the vessels perfusing the alveolus and a redirection of blood flow to other regions of lung that are better ventilated and have higher oxygen levels. This phenomenon maximizes gas exchange by matching ventilation and perfusion within the lung.
Specialized Functions: Cells Lining the Alveoli β
The alveoli are lined by cells that perform a number of important functions. Type I pneumocytes line the alveolus. Interspersed among these type I pneumocytes are other cells called type II pneumocytes, which produce surfactant, a substance that becomes a component of the liquid layer lining the alveolus. Surfactant plays a critical role in reducing surface forces within the alveolus, thereby contributing to the stability of the alveoli (see Chapter 3). Type II pneumocytes also absorb and recycle surfactant.
We have previously described the role of the airways in the body's defenses. Mucus traps inhaled foreign material, and the cilia push this material up to the trachea, where it can be expelled. The alveoli also play a part in defending the lungs from infection. Macrophages are found within the alveoli and ingest foreign material that has evaded the airway defenses and managed to enter the gas-exchanging units. These cells help police the alveoli against infectious agents to minimize damage to the lungs and prevent entry of these organisms into the bloodstream, where they can be carried to the rest of the body.